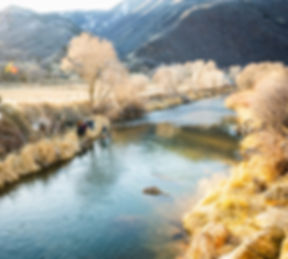
Cultivating stewardship and improving water quality in the Utah Lake watershed
Project overview
Human survival depends on access to clean water, but every year agriculture and urbanization pollute freshwater ecosystems with hundreds of millions of tons of fertilizers(1). Excess nutrients from human activity trigger toxic cyanobacteria blooms and expansive hypoxic dead zones that damage ecosystems, hurt the economy, undermine food and water security, and directly harm human health(2). This global crisis affects most freshwater and marine ecosystems(3), and is considered to be the second most urgent environmental issue, after the loss of biodiversity1. Freshwater ecosystems along the Wasatch Front have been particularly hard hit by concentrated and growing human development. Utah Lake, the largest naturally occurring freshwater lake in the western U.S., is experiencing frequent and extensive algal blooms and general impairment of ecological functioning due to nutrient overloading, altered hydrology, and climate(4). We propose to address this urgent socioecological issue by involving BYU undergraduates and community members in a large citizen-science initiative in the Utah Lake watershed. The project collaborates with local water quality organizations to involve 100 to 200 BYU undergraduates from across campus in a large synoptic sampling of water chemistry in the Provo River and Spanish Fork watersheds. By visiting and sampling their local watershed, students will deepen their understanding of the extent of human influence on ecosystems and recognize their social, spiritual, and ecological interdependence with the Earth. Data from this project will provide essential information on nutrient sources and watershed resilience, informing policy and management by the Utah Lake Steering Committee and Science Panel, overseen by the Department of Water Quality (DWQ). More broadly, our project will contribute to sustainable use of water and nutrient resources in Utah by cultivating individual stewardship and by providing critical quantitative information on an urgent socioecological issue.
Background and project goals
Nutrient pollution of freshwater and estuarine water bodies is degrading ecological functioning and ecosystem services at a global scale. Economic damage from nitrate (NO3-) contamination alone is estimated to cost 0.2 to 2.3 trillion USD annually—up to 3% of the global gross domestic product(5,6). Over the past 50 years, global fertilizer use increased by over 500%(7), and nitrogen and phosphorus pollution are expected to keep pace with population growth and meat consumption, increasing until the middle of the century(8,9). At the same time, human activity such as agriculture has disturbed approximately three-quarters of the Earth's ice-free land surface(10), reducing the capacity of ecosystems to buffer and process nutrient inputs(11–13). The resulting nutrient excess has contaminated groundwater aquifers(14–16) and increased the flux of nutrients through river systems to the sea, creating eutrophic dead zones in many lakes and estuaries, and altering biogeochemistry throughout the ocean(3,17,18). Governments have funded a broad range of programs to reduce eutrophication(19–22), but interventions have had mixed results(23–25).
Two primary factors complicate addressing eutrophication. First, nutrient transport through river networks is extremely variable in space and time due to large swings in water flow, biological activity, and human disturbance(13,26,27). Consequently, monitoring at a single station or at a single point in time cannot identify nutrient sources or quantify uptake and recycling in the river network. Second, a lack of public engagement with water quality issues reduces sociopolitical motivation to implement best practices(28,29). Many methods to improve agricultural and urban water management are known (e.g. upgrading wastewater treatment) but not widely implemented because of financial or behavioral barriers. One way to achieve scientific and societal goals is to involve non-professional community members in water quality monitoring(30). Citizen science can extend scientific observation and fundamentally change public awareness and mentality, which subsequently influences how water resources are managed(28,29). In this sense, participatory water quality monitoring is not only a means of generating understanding of how water and nutrients propagate through catchments; it is a mechanism to improve water quality itself.
Both scientific and social factors are at play in the struggle to protect and restore Utah Lake and its tributaries. It is estimated that wastewater treatment plants and diffuse nutrient sources in the watershed deliver approximately 300 tons of phosphorus annually to Utah Lake(4), though these numbers are hotly contested. Phosphorus is believed to be the proximate cause of the toxic cyanobacteria blooms that regularly affect much of the lake(31). While the phosphorus outflow from the seven wastewater treatment plants discharging into Utah Lake are closely monitored, the timing and amount of diffuse phosphorus inputs from elsewhere in the watershed are poorly understood. These diffuse nutrient sources include agricultural return flow, urban runoff, and natural geologic sources associated with rock weathering.
To address both the scientific and social obstacles to improving water quality in the Utah Lake watershed, we propose a series of large-scale sampling campaigns to capture water chemistry in the Provo and Spanish Fork Rivers, which contribute the majority of diffuse phosphorus(4). These synoptic samplings will be carried out by BYU undergraduates and community members in Utah Valley, producing an immense and rich data set (100-500 sites) and fostering a mentality of stewardship for the freshwater systems that sustain our communities. Specifically, the two central goals of the project are:
1. Increase awareness about water quality and the ecosystems that support human activity along the Wasatch Front through experiential learning.
2. Analyze the spatial and temporal variability in water chemistry using repeat synoptic sampling to identify nutrient sources, quantify how stable their locations are through time, and assess what stream and catchment characteristics most affect ecosystem resilience to nutrient loading.
Proposed work
Student and community involvement
During the high-flow period in the early spring and the low-flow period in the early fall of 2018, we will organize a synoptic sampling of 100-300 sites in the river networks of the Provo and Spanish Fork watersheds (experimental approach detailed in the next section). The experimental design and outreach strategy will be developed as a part of the Soil and Water Quality Lab (PWS 306), which will be taught by Dr. Abbott in the winter semester. The 25 students in PWS 306 will collaborate with DWQ and Utah Water Watch (UWW), a well-established citizen science program associated with the Utah State University Water Quality Extension, to select sampling sites, develop training materials, and conduct outreach to the broader campus community. This project will be the central focus of the lab, providing experiential learning opportunities surrounding the scientific method, societal engagement, quantitative ecohydrology, and environmental stewardship. To assure timely progress and to coordinate the in-class efforts of the students in PWS 306, two undergraduate students will be hired as project coordinators.
Starting in January 2018, we will conduct outreach to all BYU colleges and leverage students’ personal social networks to recruit water-sampling volunteers. We will develop an online interface allowing volunteers to create a profile and adopt one or more river-sampling sites. To improve engagement and motivation, participants will earn online badges and awards for completing training, recruiting others, and performing sampling. We will conduct a training meeting prior to each synoptic sampling, where volunteers will learn about water quality issues in Utah Valley, learn to sample safely and effectively, and pick up their sampling kits. To minimize user error, the simplified sampling protocol will consist of filtering a small volume of stream water (60 mL) into a plastic bottle and taking a picture of the site (automatic geotagging of the photo by participants’ smart phones will allow verification of sample location). On the day of each sampling, volunteers will travel to their location/s to collect and filter a water sample between 9 a.m. and 4 p.m. Samples will be returned to one of several pickup locations, staffed by students in PWS 306. Following the UWW model, all participants will be entered into a drawing for small prizes (e.g. T-shirts, hats, water bottles) after both samplings and data will be reported to the whole group. We will involve campus clubs (e.g. Environmental Science and LDS Earth Stewardship) and create a “#SaveUtahLake” hashtag to tag pictures and other posts on social networks, encouraging a sense of community and fun. We will invite motivated participants from all colleges to assist in subsequent data processing, interpretation, and presentation. Depending on interests and skills, students will participate in quantitative analysis (e.g. sample processing, spatial analysis, historical reconstruction of time series); creation of non-technical articles, blogs, and works of art connected to water quality; and local and national advocacy.
Ecohydrologic approach to locate nutrient sources and quantify catchment resilience
We will implement a new approach informed by landscape ecology and catchment hydrology for analyzing spatial and temporal variance of water chemistry in stream networks(32). We use a spatially dense sampling of solute and particulate concentrations in the river’s main stem and tributaries to calculate a distributed mass balance of nutrients and other parameters (Fig. 1). By sampling sites multiple times across a diversity of hydrologic flows, we can identify nutrient sources, quantify how stable their locations are through time, and assess what stream and catchment characteristics most affect resilience to nutrient loading (removal or retention capacity). We further constrain the relative importance of different nutrient sources (e.g. urban, agricultural, geologic) and the role of biogeochemical removal (e.g. uptake by plants and microorganisms, sorption to sediment, complexation with organic matter) with concentration-discharge relationships and biogeochemical tracers, including optical properties of dissolved organic matter; isotopes of water, nutrients, and other solutes; and elemental ratios of various ions(33). We will expand on the existing observational network maintained by DWQ, UWW, and the NSF-funded iUtah project to leverage existing data and contribute to broader efforts to understand eutrophication in Utah Lake. To complement the periodic, synoptic sampling described above, we will install high-frequency sensors near the mouths of the Provo and Spanish Fork Rivers to quantify total carbon and nutrient loads and partition contributions from spring runoff, stormflow, agricultural return flow, and groundwater. We will report results to the Utah Lake Steering Committee and Science Panel, which will inform management of the Utah Lake Watershed. We will also submit a paper to an international journal, contributing to the currently sparse peer-reviewed literature on Utah Lake.
By building on current efforts and connecting students with their watershed, this work will have a large impact during and after the funding period. It will increase awareness of water quality issues in Utah, while generating urgently needed data to address pressing societal issues. A large number of BYU students will have experiential learning opportunities that cultivate personal stewardship and empower individual and communal action.
Key collaborators
Scott Daly
-
Environmental Scientist in the Watershed Protection Section, Utah Division of Water Quality
Ellen Bailey
-
Water Quality Program Coordinator, USU Water Quality Extension
Nancy Mesner
-
Extension Water Quality Specialist, Department of Watershed Sciences, Utah State University
Eric Ellis
-
Utah Lake Commission Executive Director
References:
1. Steffen, W. et al. Planetary boundaries: Guiding human development on a changing planet. Science 347, 1259855 (2015).
2. Brooks, B. W. et al. Are harmful algal blooms becoming the greatest inland water quality threat to public health and aquatic ecosystems? Environ. Toxicol. Chem. 35, 6–13 (2016).
3. Diaz, R. J. & Rosenberg, R. Spreading Dead Zones and Consequences for Marine Ecosystems. Science 321, 926–929 (2008).
4. PSOMAS. Utah Lake TMDL: Pollutant Loading Assessment and Designated Beneficial Use Impairment Assessment. 88 (State of Utah Division of Water Quality, 2007).
5. Bodirsky, B. L. et al. Reactive nitrogen requirements to feed the world in 2050 and potential to mitigate nitrogen pollution. Nat. Commun. 5, (2014).
6. Our nutrient world: the challenge to produce more food and energy with less pollution ; [global overview on nutrient management]. (Centre for Ecology & Hydrology, 2013).
7. Foley, J. A. et al. Solutions for a cultivated planet. Nature 478, 337–342 (2011).
8. Canfield, D. E., Glazer, A. N. & Falkowski, P. G. The Evolution and Future of Earth’s Nitrogen Cycle. Science 330, 192–196 (2010).
9. Seitzinger, S. P. et al. Global river nutrient export: A scenario analysis of past and future trends. Glob. Biogeochem. Cycles 24, GB0A08 (2010).
10. Ellis, E. C., Klein Goldewijk, K., Siebert, S., Lightman, D. & Ramankutty, N. Anthropogenic transformation of the biomes, 1700 to 2000: Anthropogenic transformation of the biomes. Glob. Ecol. Biogeogr. 586–606 (2010). doi:10.1111/j.1466-8238.2010.00540.x
11. Pinay, G. et al. Upscaling Nitrogen Removal Capacity from Local Hotspots to Low Stream Orders’ Drainage Basins. Ecosystems 18, 1101–1120 (2015).
12. Seitzinger, S. et al. Denitrification across landscapes and waterscapes: a synthesis. Ecol. Appl. 16, 2064–2090 (2006).
13. Thomas, Z., Abbott, B. W., Troccaz, O., Baudry, J. & Pinay, G. Proximate and ultimate controls on carbon and nutrient dynamics of small agricultural catchments. Biogeosciences 13, 1863–1875 (2016).
14. Aquilina, L. et al. Nitrate dynamics in agricultural catchments deduced from groundwater dating and long-term nitrate monitoring in surface‐ and groundwaters. Sci. Total Environ. 435–436, 167–178 (2012).
15. Ben Maamar, S. et al. Groundwater Isolation Governs Chemistry and Microbial Community Structure along Hydrologic Flowpaths. Front. Microbiol. 6, 1457 (2015).
16. Jasechko, S. et al. Global aquifers dominated by fossil groundwaters but wells vulnerable to modern contamination. Nat. Geosci. 10, 425–429 (2017).
17. Howarth, R. W. Coastal nitrogen pollution: A review of sources and trends globally and regionally. Harmful Algae 8, 14–20 (2008).
18. Reed, D. C. & Harrison, J. A. Linking nutrient loading and oxygen in the coastal ocean: A new global scale model. Glob. Biogeochem. Cycles 30, 2015GB005303 (2016).
19. Andreen, W. L. Water Quality Today - Has the Clean Water Act Been a Success? (Social Science Research Network, 2004).
20. Hering, D. et al. The European Water Framework Directive at the age of 10: A critical review of the achievements with recommendations for the future. Sci. Total Environ. 408, 4007–4019 (2010).
21. Liu, J. & Yang, W. Water sustainability for China and beyond. Science 337, 649–650 (2012).
22. Withers, P. J. A. & Haygarth, P. M. Agriculture, phosphorus and eutrophication: a European perspective. Soil Use Manag. 23, 1–4 (2007).
23. Jarvie, H. P. et al. Phosphorus Mitigation to Control River Eutrophication: Murky Waters, Inconvenient Truths, and “Postnormal” Science. J. Environ. Qual. 42, 295–304 (2013).
24. Jenny, J.-P. et al. Urban point sources of nutrients were the leading cause for the historical spread of hypoxia across European lakes. Proc. Natl. Acad. Sci. 201605480 (2016). doi:10.1073/pnas.1605480113
25. Wilcock, R. J. et al. Trends in water quality of five dairy farming streams in response to adoption of best practice and benefits of long-term monitoring at the catchment scale. Mar. Freshw. Res. 64, 401–412 (2013).
26. Gascuel-Odoux, C., Aurousseau, P., Durand, P., Ruiz, L. & Molenat, J. The role of climate on inter-annual variation in stream nitrate fluxes and concentrations. Sci. Total Environ. 408, 5657–5666 (2010).
27. Moatar, F., Meybeck, M., Raymond, S., Birgand, F. & Curie, F. River flux uncertainties predicted by hydrological variability and riverine material behaviour. Hydrol. Process. 27, 3535–3546 (2013).
28. Linton, J. Modern water and its discontents: a history of hydrosocial renewal. Wiley Interdiscip. Rev. Water 1, 111–120 (2014).
29. Schmidt, J. J. Historicizing the hydrosocial cycle. Water Altern. 7, (2014).
30. Bonney, R. et al. Next Steps for Citizen Science. Science 343, 1436–1437 (2014).
31. Strong, A. E. Remote sensing of algal blooms by aircraft and satellite in Lake Erie and Utah Lake. Remote Sens. Environ. 3, 99–107 (1974).
32. Abbott, B. W. et al. Unexpected stability and synchrony of water quality in stream networks. Ecol. Lett. (2017). doi:10.1111/ele.12897
33. Abbott, B. W. et al. Using multi-tracer inference to move beyond single-catchment ecohydrology. Earth-Sci. Rev. 160, 19–42 (2016).

Figure 1. Example of how synoptic sampling of stream chemistry can reveal the strength and location of solute sources and sinks. A) The distribution of three hypothetical solute sources and sinks is represented by shading, where darker patches are strong net sources (100) and lighter patches make no net contribution (0). B) Simulated solute concentrations at the sampling points plotted against subcatchment size. Though the three solutes have the same concentration at the catchment outlet, differences in source patch size alter the location of the downstream collapse of spatial variance, represented by the vertical colored bars. C) The leverage or influence of a subcatchment on outlet chemistry depends on subcatchment discharge and difference from the outlet concentration. On an applied level, knowing the patch size and location of solute sources and sinks allows targeted restoration interventions in subcatchments exerting a disproportionate influence on flux at the catchment outflow.